Tolerance of two anhydrobiotic tardigrades Echiniscus testudo and Milnesium inceptum to hypomagnetic conditions
Author and article information
Abstract
The open space is a hostile environment for all lifeforms not only due to vacuum, high radiation, low atmospheric pressure, and extremely low temperature, but also the absence of the geomagnetic field. The geomagnetic field protects Earth mainly from corpuscular radiation, that is, solar wind and cosmic radiation, but above all it influences organisms, including their cells, tissues and organs. Moreover, numerous studies conducted on plants and animals confirmed that hypomagnetic conditions (the term referring to all situations when the magnetic field is weaker than the typical geomagnetic field) have significant influence on the metabolism of living organisms. Although many studies dealt with a variety of aspects related mainly to the influence of hypomagnetic conditions on human health. Very few studies have considered the influence of hypomagnetic conditions on extremophiles. Astrobiologists have long been testing different extremofiles to find out if any multicellular organisms are able to survive in extreme conditions of open space. Among all multicellular extremophiles fit for such research, water bears (Tardigrada) are the most interesting. Not only are they one of the most resistant organisms on Earth, but results obtained from studies on these invertebrates can be extrapolated or applied to vertebrates (including humans). Despite this, studies on the influence of hypomagnetic conditions on tardigrades are rare, so far. In the present study, to test the influence of hypomagnetic conditions on the process of anhydrobiosis while entering and returning from anhydrobiosis, we used two terrestrial anhydrobiotic species that are Echiniscus testudo and Milnesium inceptum. To exclude the ambient magnetic field, experiments were carried out in a special magnetic field shielding chamber. In total, three experiments were conducted: (a) tardigrades in anhydrobiosis, (b) tardigrades entering anhydrobiosis and (c) tardigrades returning to active life. The obtained results clearly showed that even partial isolation from the geomagnetic field, that is, hypomagnetic conditions, has negative influence on anhydrobiotic abilities of both tested tardigrade species. In both species we observed lower survivability rate while entering anhydrobiosis, in anhydrobiotic state and returning to the active state. What is more, we observed a higher mortality rate in Ech. testudo than Mil. inceptum which suggest that different species response to the hypomagnetic conditions in different way. In conclusion, while current knowledge on the influence of hypomagnetic conditions on mortality of invertebrates is very limited, our results suggest that the presence of the magnetic field is a very important factor which should be considered in further research focused on potential survival of Earth organisms in outer space, spacecrafts or different planets and moons.
Cite this as
2021. Tolerance of two anhydrobiotic tardigrades Echiniscus testudo and Milnesium inceptum to hypomagnetic conditions. PeerJ 9:e10630 https://doi.org/10.7717/peerj.10630Main article text
Introduction
Outer space is a hostile environment for all lifeforms not only due to vacuum, high radiation, low atmospheric pressure, and extremely low temperature (Chuss, 2008), but also the absence of the geomagnetic field (Hargreaves, 1995; Sibeck & Lin, 2014). Astrobiologists have long been testing different organisms to find out if any multicellular organisms are able to survive in such extreme conditions (Langbein, 1986; Gmünder et al., 1989; Morrow et al., 1994). These studies are important from two points of view: first, it is important to identify organisms that are able to survive in unfavourable conditions, for example, on different planets or moons; second, we need to identify organisms which can potentially contaminate space probes sent to other celestial bodies and subsequently falsify research on search for extra-terrestrial life (Caplin, 2019; Vidaurri, 2019). Discussion focusing on interplanetary contamination, that is, the risk of transferring different lifeforms or organic material to an explored celestial body, are not new as it started in the late 1950s (National Research Council, 2006). As a result, broad recommendations for planetary protection were included in Article IX of the United Nations Outer Space Treaty from 1967 (National Research Council, 2006). Moreover, the Committee on Space Research (COSPAR) prepared strict recommendations for the sterilisation of spacecraft, landers and rovers (Committee on Space Research (COSPAR), 2002; National Research Council, 2006). Even if such distant missions with living organisms on-board, also extremophiles, have been rare, but we cannot exclude that they will be planned in the future (Erdmann & Kaczmarek, 2017). Still, despite many doubts and potential problems, we cannot abandon missions involving living organisms, because they are too important in the context of future space exploration. Studies conducted on cells, tissues and entire living organisms (active or cryptobiotic) are crucial for the future of manned space missions to Mars and other locations in the Solar System. For such missions, we need to know the limits within which living organisms can survive. What is more, such knowledge is necessary to define the probability of finding extraterrestrial life.
Among all multicellular extremophiles fit for such research, tardigrades are the most interesting. Not only are they one of the most resistant organisms on Earth (Copley, 1999), but results obtained from studies on these invertebrates can be extrapolated or applied to vertebrates, including humans (Hashimoto et al., 2016).
Tardigrada or water bears are microinvertebrates (mean size ca. 500 μm) which inhabit almost all terrestrial and aquatic ecosystems throughout the world (Nelson, Guidetti & Rebecchi, 2015). On Earth, in laboratory conditions tardigrades are able to survive many physical and chemical stressors, such as low and high temperatures, high doses of radiation, drying, low and high atmospheric pressure, low gravity, high concentration of heavy metals, toxins like CO2 or ethidium bromide (see reviews, for example, Ramlov & Westh, 2001; Altiero et al., 2011; Guidetti et al., 2012; Erdmann & Kaczmarek, 2017; Kaczmarek et al., 2019). However, in the context of astrobiology, the most important question is if tardigrades are able to survive in all these stress conditions also in extraterrestrial environments. Although it was shown that some tardigrades are able to survive even the exposure to the space vacuum (Jönsson et al., 2008; Rebecchi et al., 2009a, 2009b; Persson et al., 2011; Rebecchi et al., 2011a, 2011b; Vukich et al., 2012), the experiments were conducted within the protection of Earth’s magnetic field, i.e. on the low Earth orbit (ESA, official materials for FOTON-M3 Mission, http://esamultimedia.esa.int/docs/foton/FOTON-M3_brochure.pdf).
The magnetic field is absent or much weaker in the outer space and on some planets and moons, such as Venus, Mars, the Moon, which do not generate their own inner magnetic field. Although this fact has been known for a long time, the absence of the geomagnetic field has been ignored in all astrobiological experiments conducted on tardigrades and other extremophiles. The term ‘hypomagnetic conditions’ refers to all situations when the magnetic field is weaker than the typical geomagnetic field (GMF), including its complete absence, which is one of characteristics of the outer space outside Earth’s magnetosphere. Due to the solar wind, Earth’s magnetosphere is asymmetrical and it reaches ca. 65,000 km (10 Earth’s Radius) from Earth’s surface on the side faces the Sun (Hargreaves, 1995); whereas on Earth’s nightside, the magnetic field extends into magnetotail, measuring from 6.3 × 105 km (100 Earth’s Radius) in the near-magnetotail to ca.12.6 × 105 km in the distant magnetotail (Sibeck & Lin, 2014). The GMF protects Earth mainly from corpuscular radiation, that is, solar wind and cosmic radiation, but above all it influences organisms, including their cells, tissues and organs (Dubrov, 1978; Conley, 1970; Tombarkiewicz, 2008; Janicki, 2008; Baek et al., 2019). The GMF is also a source of information for many organisms (mainly animals) whose abilities include magneto-detection and magneto-navigation (Wiltschko & Wiltschko, 2005; Brothers & Lohmann, 2015; Yosef et al., 2020). That is why it comes as no surprise that hypomagnetic conditions have a strong negative impact on Earth’s organisms (Dubrov, 1978; Conley, 1970; Janicki, 2008; Tombarkiewicz, 2008).
Studies on organisms’ tolerance to long-term isolation from Earth’s magnetic field are particularly important for astrobiology and space medicine (Dubrov, 1978; Grigoriev & Potapov, 2014). Their heyday was in the 1970s when the influence of hypomagnetic conditions on living organisms was analysed, including space flight conditions (Conley, 1970; Dubrov, 1978). In those and later studies researchers dealt with a variety of aspects related mainly to the influence of hypomagnetic conditions on human health (Dubrov, 1978; Binhi & Sarimov, 2013; Mo, Liu & He, 2014; Mo et al., 2014; Gurfinkel et al., 2016) or on animal’s metabolism (mostly mice and rats) (Conley, 1970; Tombarkiewicz, 2008). All of these studies clearly demonstrated that a long-term exposure to hypomagnetic conditions had a significant influence on the tested organisms, reducing their fitness and activity (Conley, 1970; Tombarkiewicz, 2008; Ding et al., 2018), hypomagnetic conditions also affected the functioning of the brain (Zaporozhan et al., 2002; Mo, Liu & He, 2014; Wei-Chuan et al., 2015 ), that is, in humans by deteriorating cognitive abilities (Sarimov, Binhi & Milyaev, 2008; Binhi & Sarimov, 2009; Binhi, 2012). Moreover, long-term exposition to hypomagnetic conditions leads to circadian rhythm disorder (Gurfinkel et al., 2016), decreased metabolism, gastrointestinal diseases, and a decreased number of leukocytes (Janicki, 2008). Embryonic development was negatively affected because of inhibited early embryogenesis and reproduction capacity (Wang et al., 2003; Zhang et al., 2004; Osipenko et al., 2008; Xiao et al., 2009; Fesenko et al., 2010). Although studies on the influence of hypomagnetic conditions on humans and other vertebrates have been quite common, similar attention to invertebrates is still scarce (Dubrov, 1978).
So far, studies on the influence of hypomagnetic conditions on tardigrades are limited to only one paper published by Erdmann et al. (2017). A research on eutardigrade Hypsibius dujardini (Doyère, 1840) (recently redescribed under the name Hys. exemplaris Gąsiorek et al., 2018 and differentiated from Hys. dujardini sensu stricto) shows that in hypomagnetic conditions its mortality is much higher while entering anhydrobiosis or returning to the active state (Erdmann et al., 2017). On the basis of the obtained results, a hypothesis was formed that certain metabolic processes associated with anhydrobiosis could be disturbed or impaired by hypomagnetic conditions, for example some changes appeared in the expression of stress proteins, but the results were not conclusive (Erdmann et al., 2018). Besides, Hys. exemplaris used in those studies was not the best candidate for testing its anhydrobiotic abilities, because as an aquatic species its ability to survive in anhydrobiotic conditions is low (Wright, 1989; Kondo, Kubo & Kunieda, 2015; Boothby et al., 2017; Boothby, 2018).
In the present study, to test the influence of hypomagnetic conditions on the process of anhydrobiosis while entering and returning from anhydrobiosis, we used terrestrial tardigrade species, such as Heterotardigrada Echiniscus testudo (Doyère, 1840) and Eutardigrada Milnesium inceptum Morek et al. (2019), whose anhydrobiotic abilities are very high (Wright, 1989; Jönsson, Borsari & Rebecchi, 2001; Rebecchi et al., 2006; Jørgensen, Møbjerg & Kristensen, 2007; Jørgensen et al., 2013; Roszkowska et al., 2018). We studied meticulously how hypomagnetic conditions affected survivability of both terrestrial species at their different physiological stage (active vs in anhydrobiosis) and then compared the results with results obtained for aquatic Hys. exemplaris.
Materials and Methods
Samples processing
Two tardigrade species were collected in a xerothermic habitat that is, mosses on concrete wall in the city centre of Poznań in Poland (Heliodor Święcicki Clinical Hospital of Poznań University of Medical Sciences at Przybyszewskiego street, 52°24′15″N, 16°53′18″E; 87 m asl). Tardigrades were obtained from samples according to a standard procedure (Stec et al., 2015). Moss samples were placed in plastic beakers containing 250 ml of spring water (Żywiec Zdrój) mixed with distilled water (in ratio 1:3), called the medium. After 24 h, the mosses were strongly shaken with tweezers and all plant particles were removed. The water containing tardigrades was poured into a 250 ml plastic cylinder. After 30 min the upper portion of water (ca. 200 ml) was decanted, and the remaining 50 ml was poured into Petri dishes, while living tardigrades were extracted under a stereomicroscope (Olympus SZ51) and transferred to small Petri dishes with clean water.
Model species
Echiniscus testudo is a widely distributed parthenogenetic, herbivorous and terrestrial heterotardigrade species (McInnes, 1994; Jørgensen, Møbjerg & Kristensen, 2007; Jørgensen et al., 2013; Kaczmarek, Michalczyk & McInnes, 2015, 2016; McInnes, Michalczyk & Kaczmarek, 2017; Gąsiorek, Vončina & Michalczyk, 2019), while Mil. inceptum is a dioecious (but it can also reproduce parthenogenetically), predatory and terrestrial Eutardigrade species known from few distant localities (Roszkowska et al., 2018; Morek et al., 2019). Both species inhabit very dry xerothermic mosses or/and lichens. Due to the fact that the methodology for culturing Heterotardigrada is not known, specimens of Ech. testudo were extracted directly from the samples. They were kept two additional hours in a clean water and then these specimens were segregated and only fully active adult specimens of medium body length were selected for experiments. Fully active specimens of Mil. inceptum were extracted directly from the sample and a laboratory culture was established. Parthenogenetic Milnesium females were cultured in the medium on scratched Petri dishes where rotifers (Lecane inermis Bryce, 1892) and nematodes (Caenorhabditis elegans Maupas, 1900) were added ad libitum as a food source. Fully active, adult and not moulting females of medium body size were selected for experiments. Before experiments, the chosen tardigrades were kept two additional hours in clean water.
Anti-magnetic chamber and control box
To reduce the ambient magnetic field, all experiments were carried out inside a special magnetic field shielding chamber (Chamber Isolated from Magnetic Field—CIMF) constructed and used by our team in our previous studies on Hys. exemplaris (Erdmann et al., 2017). The CIMF is a double cylinder closed at both ends with a double-layered lid, with internal size of 26.2 cm of diameter and 35 cm of length (Fig. 1B), made of a mu-metal (μ-metal) tinware (for more details see Erdmann et al., 2017). Like some other iron or nickel-based amorphous and/or nanocrystalline alloys with Zr, Nb or Mo additions (Kopcewicz et al., 1999; Miglierini et al., 2002; Idzikowski et al., 2004), mu-metal is easily saturated in static or slowly varying magnetic fields, which is appropriate for use as a shielding material.
Figure 1: Chamber Isolated from the Magnetic Field (CIMF).
(A) CIMF chamber during work, connected with a laptop, (B) inside view of the CIMF chamber, with shelves for test objects. The internal size of CIMF is 26.2 cm in diameter and 35 cm in length.The geomagnetic field inside and outside the chamber and the temperature inside the CIMF were measured and later transmitted for analyses through a USB interface to the computer (for more details see Erdmann et al., 2017) (Fig. 1A).
Following the same protocol as in our previous work (Erdmann et al., 2017) to provide conditions similar to those inside the CIMF, but without hypomagnetic condition, for control groups, the Control Box was used. As the Control Box is a plastic container of the size of 18.5 × 12 × 33 cm, uninsulated from the magnetic field.
Experiments methodology
To test the influence of hypomagnetic conditions on the process of anhydrobiosis of Ech. testudo and Mil. inceptum, three experiments were conducted: (I) with specimens in anhydrobiosis, (II) with specimens entering anhydrobiosis, and (III) with specimens returning to active life.
In every experiment 200 specimens (100 in the control group and 100 in the test group) of both species were used. In each experiment, 10 replicates were performed, with 10 specimens in each Petri dish (both in control and test groups).
All selected animals were placed in plastic Petri dishes (ø = 40 mm) on filter paper (grammage 85–87 gsm, Chemland Company) moistened with 450 microliters of the medium (spring water “Żywiec Zdrój” mixed with distilled water in ratio 1:3). Dehydration of all specimens took 3 days in the average temperature of 23.7°C (Data S1). As we explained in our previous work “Can the tardigrade Hypsibius dujardini survive in the absence of the geomagnetic field?” additional monitoring equipment (i.e., electronic hygrometers) cannot be used as it could increase the level of the magnetic field in the chamber. Therefore, humidity level inside CIMF and Control Box was not controlled nor modified (Erdmann et al., 2017). Air humidity in both CIMF and Control Box was approximately the same as in the laboratory and range between 40% and 60%.
Because we wanted our newest results to be comparable to the results of our older studies on Hys. exemplaris (Erdmann et al., 2017) we adapted as much as possible procedures used in those earlier studies. All experiments were conducted for 21 days in the same laboratory room. Test groups were kept either in the CIMF or—when necessary (when tested specimens should be kept in normal magnetic conditions)—in a box that was identical to the Control Box. Control groups were kept in the Control Box. However, a different procedure for rehydration was used, because the newly tested species do not need as long rehydration time as, previously used Hys. exemplaris. Rehydration was conducted, for 1 h, in the 450 microliters medium which was added to dried Petri dishes. After this time, specimens were observed continuously for the following 2 h, with three hours in total time spent, by specimens, in the medium Later, specimens were divided into dead (without signs of movements of the body, legs or internal structures) or alive (with at least some signs of movements).
To measure the rate of tardigrade mortality (expressed as the percentage of dead individuals) in all experiments both dead and specimens were counted and typed into databases, first one for Ech. testudo (Data S2), and second for Mil. inceptum (Data S3).
Experiment I. In this experiment, we tested the influence of hypomagnetic conditions on anhydrobiotic individuals of Ech. testudo and Mil. inceptum. The experiment was conducted as previously described in Erdmann et al. (2017). Specifically, specimens from test groups were dehydrated on Petri dishes and later placed in the CIMF for 21 days. After this time, they were removed from the chamber and rehydrated on the same Petri dishes. Concurrently, the dehydrated specimens from the control group spent 21 days in the Control Box. After this time, all specimens from both the CIMF and the Control Box were rehydrated and living and dead specimens from both groups were counted.
Experiment II. In this experiment we, tested the influence of hypomagnetic conditions on the process of entering anhydrobiosis. Following the procedure form our previous work (Erdmann et al., 2017) specimens in the tested groups were dehydrated inside the CIMF, and then Petri dishes with tardigrades were removed from the chamber. The specimens spent 21 days in this state under the influence of the geomagnetic field in the Control Box in the same conditions as the control group. The specimens from the control group were dehydrated in the geomagnetic field, and then spent 21 days in the Control Box. After this period, all specimens were rehydrated in normal geomagnetic field conditions. Living and dead specimens from both groups were counted.
Experiment III. In this experiment, we tested the influence of hypomagnetic conditions on tardigrades in the process of returning from anhydrobiotic to the active state. The experiment was conducted as previously described in Erdmann et al. (2017). Specifically, specimens of both tested species were dehydrated outside the CIMF and spent 21 days in the anhydrobiotic state under the influence of the geomagnetic field. Subsequently, Petri dishes with tested specimens were transferred to the CIMF, and specimens were rehydrated in the medium in hypomagnetic conditions Specimens from the control group spent 21 days after dehydration in the Control Box, and then were rehydrated in the same conditions. After rehydration, all specimens were rehydrated and living and dead specimens from both groups were counted.
Statistical analysis
In all three experiments, tardigrade mortality in each Petri dish was calculated (Datas S2 and S3). To test the differences in mortality between both groups, that is, experimental vs control, we used the t-test with the Cochran-Cox adjustment, whereas differences between particular experiments were tested with the one-way ANOVA (Zar, 1999) with the Tukey test as a post-hoc. In the latest case, the control group is expressed as the minimum mortality on each dishes for each control groups from three experiments (mean ± SE of Ech. testudo = 9.0 ± 2.3, mean ± SE of Mil. inceptum = 7.0 ± 2.1). All calculations were performed with the R 3.2.3.
To check whether mortality of the tested tardigrade species did not fluctuate even in normal magnetic field conditions, the one-way ANOVA test was carried out for test groups of both species alike. No significant differences were found in mortality of control groups in all three experiments for either Ech. testudo (one-way ANOVA: F2,27 = 0.97, p = 0.39) or Mil. inceptum (F2,27 = 1.25, p = 0.21).
Results
Experiment I: testing the influence of hypomagnetic conditions on anhydrobiotic individuals
The mean (±SE) percentage of mortality in experimental groups was 46.00% (±5.41%) and 32.00% (±3.88%) for Ech. testudo and Mil. inceptum, respectively. On the other hand, mortality in control groups was clearly lower and it amounted to 29.00% (±4.33%) for Ech. testudo (Table 1) and 21.00% (±3.48%) for Mil. inceptum (Table 1). The differences were statistically significant (Ech. testudo: t17 = 2.45, p = 0.025, Fig. 2A, and Mil. inceptum: t17 = 2.18, p = 0.045, Fig. 3A).
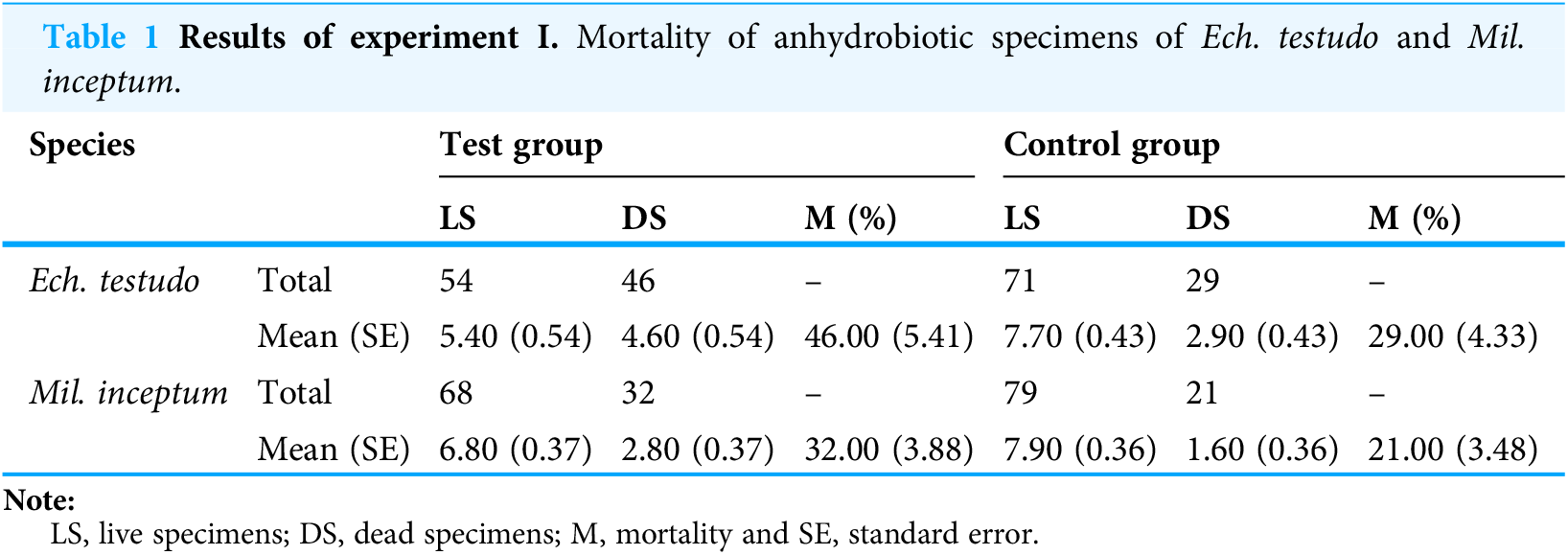
Species | Test group | Control group | |||||
---|---|---|---|---|---|---|---|
LS | DS | M (%) | LS | DS | M (%) | ||
Ech. testudo | Total | 54 | 46 | – | 71 | 29 | – |
Mean (SE) | 5.40 (0.54) | 4.60 (0.54) | 46.00 (5.41) | 7.70 (0.43) | 2.90 (0.43) | 29.00 (4.33) | |
Mil. inceptum | Total | 68 | 32 | – | 79 | 21 | – |
Mean (SE) | 6.80 (0.37) | 2.80 (0.37) | 32.00 (3.88) | 7.90 (0.36) | 1.60 (0.36) | 21.00 (3.48) |
Note:
LS, live specimens; DS, dead specimens; M, mortality and SE, standard error.
Figure 2: Results of experiments on Echiniscus testudo Doyère, 1840 shown as comparisons of mortalities in test and control groups.
The diagrams show (A) comparisons of mortalities of anhydrobiotic specimens, (B) comparisons of mortalities of the specimens during entering into cryptobiosis, (C) comparisons of mortalities of the specimens returning to an active state, and (D) the comparison of experiments. Every diagram shows the difference in mortality (expressed as the percentage of dead individuals) between the Test Group and the Control Group. Blue indicates statistically significant differences, while gray indicates statistically insignificant differences. Max, maximal value; Min, minimal value; Mean, mean value and SE, stands for standard error.Figure 3: Results of experiments on Milnesium inceptum Morek et al., 2019, shown as comparisons of mortalities in test and control groups.
The diagrams show (A) comparisons of mortalities of anhydrobiotic specimens, (B) comparisons of mortalities of the specimens during entering into cryptobiosis, (C) comparisons of mortalities of the specimens returning to an active state, and (D) the comparison of experiments. Every diagram shows the difference in mortality (expressed as the percentage of dead individuals) between the Test Group and the Control Group. Blue indicates statistically significant differences, while gray indicates statistically insignificant differences. Max, maximal value; Min, minimal value; Mean, mean value and SE, standard error.Experiment II: testing the influence of hypomagnetic conditions on tardigrades in the process of entering anhydrobiosis
In this case, the mean (±SE) percentage of mortality in experimental groups was 38.00% (±6.11%) for Ech. testudo (Table 2) and 33.00% (±5.97 %) for Mil. inceptum (Table 2). Again, mortality in control groups was clearly lower 24.00% (±5.20%) for Ech. testudo (Table 2) and 12.00% (±3.26 %) for Mil. inceptum (Table 2). However, the differences were statistically significant only for Mil. inceptum (t17 = 3.08, p = 0.008, Fig. 2B), and not for Ech. testudo (t17 =1.74, p = 0.09, Fig. 3B).
Species | Test group | Control group | |||||
---|---|---|---|---|---|---|---|
LS | DS | M (%) | LS | DS | M (%) | ||
Ech. testudo | Total | 62 | 38 | – | 76 | 24 | – |
Mean (SE) | 6.20 (0.61) | 3.80 (0.61) | 38.00 (6.11) | 7.60 (0.52) | 2.40 (0.52) | 24.00 (5.20) | |
Mil. inceptum | Total | 67 | 33 | – | 88 | 12 | – |
Mean (SE) | 6.70 (0.59) | 3.30 (0.59) | 33.00 (5.97) | 8.8 (0.32) | 1.20 (0.32) | 12.00 (3.26) |
Note:
LS, live specimens; DS, dead specimens; M, mortality and SE, standard error.
Experiment III: testing the influence of hypomagnetic conditions on tardigrades returning from anhydrobiosis to the active state
The mean (±SD) percentage of mortality in experimental groups was 59.00% (±4.06%) for Ech. testudo (Table 3) and 61.00% (±3.78%) for Mil. inceptum (Table 3). Mortality in control groups was clearly lower, that is, 20.00% (±4.47%) for Ech. testudo (Table 3) and 28.00% (±3.59%) for Mil. inceptum (Table 3). The differences were statistically significant for both Ech. testudo ( t17 = 6.45, p = 0.0000001, Fig. 2C) and Mil. Inceptum (t17 = 6.32, p = 0.0000001, Fig. 3C).
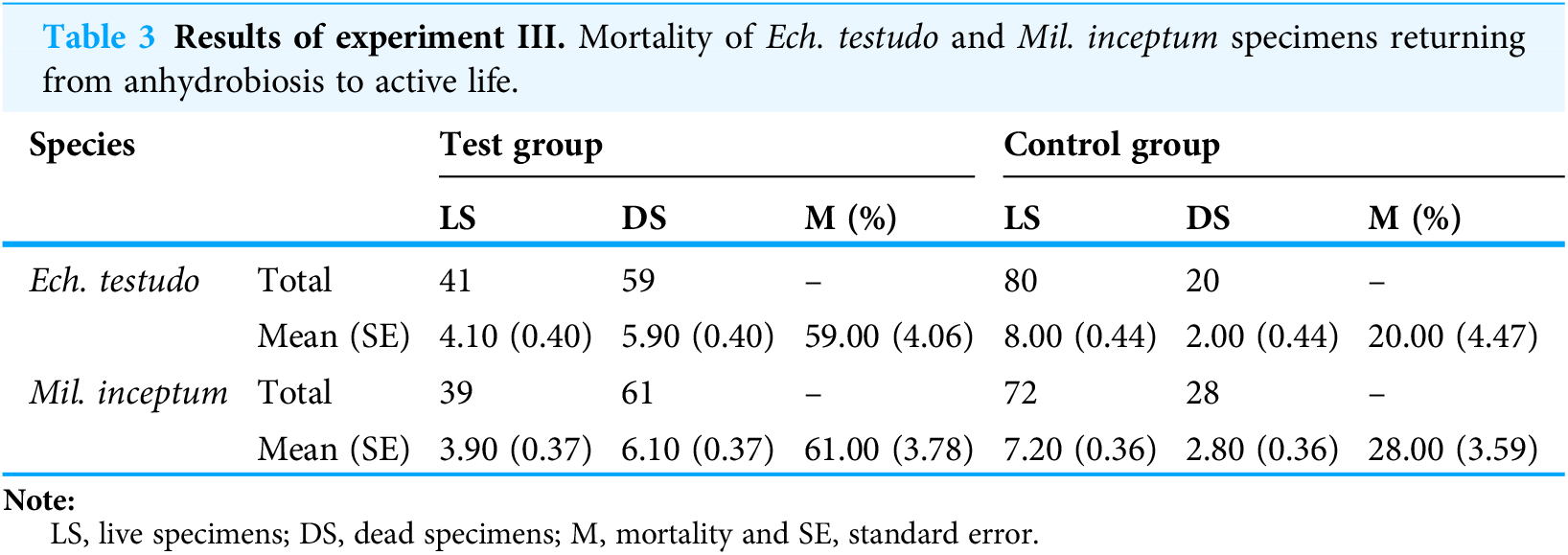
Species | Test group | Control group | |||||
---|---|---|---|---|---|---|---|
LS | DS | M (%) | LS | DS | M (%) | ||
Ech. testudo | Total | 41 | 59 | – | 80 | 20 | – |
Mean (SE) | 4.10 (0.40) | 5.90 (0.40) | 59.00 (4.06) | 8.00 (0.44) | 2.00 (0.44) | 20.00 (4.47) | |
Mil. inceptum | Total | 39 | 61 | – | 72 | 28 | – |
Mean (SE) | 3.90 (0.37) | 6.10 (0.37) | 61.00 (3.78) | 7.20 (0.36) | 2.80 (0.36) | 28.00 (3.59) |
Note:
LS, live specimens; DS, dead specimens; M, mortality and SE, standard error.
A comparison of survival rates depending on the moment of isolation from the geomagnetic field
We found differences between all three experiments for both species (Ech. testudo: one-way ANOVA F2,27 = 13.15, p = 0.00010, Mil. inceptum one-way ANOVA F2.36 = 27.96, p = 0.000000001), but there was no general pattern. According to the post-hoc Tukey test, the largest differences for Ech. testudo were observed between third experiment and the control group (post-hoc Tukey: p < 0.0000001), the second and the third experiment (post-hoc Tukey: p = 0.00001). Differences between the first experiment and the control group (post-hoc Tukey: p = 0.000005) and between the first and the second experiment (post-hoc Tukey: p = 0.006),were smaller, but still statistically significant (Fig. 2D; Data S4). On the other hand, differences for Mil. inceptum between particular experimental groups were statistically significant (in all cases post-hoc Tukey: p < 0.0001) as shown in Fig. 3D (Data S5) except the first experiment vs the second experiment as no significant differences were found (post-hoc Tukey: p = 0.99). Differences recorded between the first and the third experiment, the second and the third were equally high (post-hoc Tukey: p = 0.0001). The smallest difference was observed between the first experiment and the control group (post-hoc Tukey: p = 0.0008).
Discussion
The results obtained in experiments on heterotardigrade Ech. testudo and eutardigrade Mil. inceptum clearly showed that even partial isolation from the geomagnetic field, that is, hypomagnetic conditions, has negative influence on anhydrobiotic abilities of tardigrades. The general trend remains similar to the results obtained for Hys. exemplaris (Erdmann et al., 2017), however, significant differences in the reaction to hypomagnetic conditions were observed among representatives of different tardigrade species.
In studies conducted on Hys. exemplaris, we identified two critical moments for anhydrobiosis abilities, that is, while entering anhydrobiosis, and upon returning to the active state. Both processes were strongly affected by hypomagnetic conditions. However, no influence of hypomagnetic conditions was observed for specimens in the state of anhydrobiosis (Erdmann et al., 2017). This observation partially contradicts the results for Ech. testudo and Mil. inceptum, because both species demonstrated a negative reaction to hypomagnetic conditions not only while entering anhydrobiosis and returning to the active state, but also in the state of anhydrobiosis.
It is well known that hypomagnetic conditions affect metabolic and biochemical processes of other organisms (Dubrov, 1978; Conley, 1970; Tombarkiewicz, 2008; Janicki, 2008; Baek et al., 2019). But, due to a current knowledge tardigrade metabolism during anhydrobiosis is significantly decreased or even stopped (Pigoń & Węglarska, 1953, 1955; Clegg, 1973; Hengherr, Brümmer & Schill, 2008; Schill & Hengherr, 2018), that is connected with “Sleeping Beauty” hypothesis which assumes that during cryptobiosis tardigrades do not age (for review see Kaczmarek et al., 2019). Therefore, no differences among anhydrobiotic tardigrades in normal and hypomagnetic conditions should occur. However, even if the “Sleeping Beauty” model of anhydrobiosis is correct, and most biochemical processes, including ageing, are stopped, tardigrades are still affected by hypomagnetic conditions, due to potential cellular damage caused by chemical ageing (Schill & Hengherr, 2018). Our results can suggest that although metabolism during anhydrobiosis is probably very low, it is still probably on the level which can be affected by hypomagnetic conditions.
Results of experiments II and III obtained for Mil. inceptum are very similar to the results conducted on Hys. exemplaris (Erdmann et al., 2017), whereas results obtained for Ech. testudo were contradictory. In the case of Mil. inceptum, both experiments demonstrated that the two critical moments for this species happened upon entering anhydrobiosis (experiment II) and returning to the active state (experiment III), because hypomagnetic conditions significantly increased mortality in the test groups. In the case of Ech. testudo, we noticed significant differences between the control and test group in experiment III, and the obtained differences were even higher than for Mil. inceptum. However, differences found between the test and the control group of Ech. testudo in experiment II were not statistically significant. It can mean that Ech. testudo is less resistant to hypomagnetic conditions while returning to the active state than Mil. inceptum or Hys. exemplaris, but more resistant to hypomagnetic conditions while entering anhydrobiosis. Mortality trends in response to hypomagnetic conditions are similar for both of the studied species, and additionally Hys. exemplaris, that is, the highest mortality was observed in response to hypomagnetic conditions, but the mean mortality of Ech. testudo and Mil. inceptum was clearly lower in comparison to Hys. exemplaris (Erdmann et al., 2017). These results are connected with generally higher anhydrobiotic abilities of Ech. testudo and Mil. inceptum, which are terrestrial species inhabiting xerothermic habitats, as opposed to Hys. exemplaris which is a freshwater species with a low anhydrobiotic ability (Wright, 1989).
All these results lead to a conclusion that hypomagnetic conditions increase tardigrade mortality rate during anhydrobiosis. However, there are certain differences among different species, especially those with varying anhydrobiotic abilities and inhabiting different microhabitats.
Our research demonstrates that the influence of hypomagnetic conditions, often neglected in studies focused on potential survivability in space vacuum (for review see for example, Guidetti et al., 2012; Erdmann & Kaczmarek, 2017), should also be considered in future research on extremophiles, including tardigrades, to test their abilities to survive in the outer space or on different planets or moons. It is of highest importance, because hypomagnetic conditions significantly increase mortality of all studied tardigrade species. What else is equally important is the choice of a possible model species for further astrobiological studies. This is well visible in the case of Hys. exemplaris which is often used as a model species in different types of research (Gabriel et al., 2007; Bemm et al., 2016; Fernandez et al., 2016; Hering et al., 2016; Hyra et al., 2016; Kosztyła et al., 2016). Its low anhydrobiotic abilities and low tolerance to hypomagnetic conditions indicate that it should be replaced by other species, as results obtained in studies on Hys. exemplaris fail completely to be representative for Tardigrada.
Tardigrades are used increasingly in space research, mainly because of their high resistance to many environmental stressors (see for example, Guidetti et al., 2012; Erdmann & Kaczmarek, 2017). In the past not only astrobiologists, but also space law experts considered the risk of contaminating other celestial bodies with terrestrial life as the consequence of space exploration (Committee on Space Research (COSPAR), 2002; National Research Council, 2006).
This risk assessed in purely theoretical considerations became not so far-fetched when in 2019 an Israeli spacecraft, Beresheet, crashed on the Moon with tardigrades onboard (NASA Space Science Data Coordinated Archive, 2020; Møbjerg et al., 2020; Shahar & Greenbaum, 2020). What is more, according to COSPAR guidelines, Moon missions fall under category II, and requirements for preventing biological contamination are low and no sterilisation of the entire lander, using of clean rooms, or even inventory of organic samples is needed. What is particularly disturbing is the fact that SpaceIL, an Israeli organisation established in 2011 competing in the Google Lunar X Prize (GLXP) contest to land a spacecraft on the Moon, was unaware that tardigrades along with other biological samples had been added to a payload by the US-based Arch Mission Foundation. Nevertheless, the risk of contaminating the Moon by tardigrades was very low, because tardigrades were closed in a hermetic multi-layered metal box. Even if the container had been damaged during the collision, it would have been very unlikely for the tardigrades in the cryptobiotic state to return to activity and survive in such adverse environmental conditions, especially the lack of liquid water (Caplin, 2019). Although we do not know what happened with the tardigrades and the chances of their survival seemed small, the Beresheet spacecraft incident has sparked a new discussion on this topic (Caplin, 2019; Vidaurri, 2019; Shahar & Greenbaum, 2020), reminding us on exercising caution in space exploration. Currently, we know that at least several tardigrade species are able to survive conditions of space vacuum and possible conditions on some celestial bodies in the Solar System (for review see Guidetti et al., 2012; Erdmann & Kaczmarek, 2017; Jagadeesh, Roszkowska & Kaczmarek, 2018). Therefore, a question arises if it is possible that the influence of hypomagnetic conditions could be sufficient to completely eradicate the threat of space contamination by terrestrial extremophilic lifeforms. The response is ‘probably not’, but such a possibility might not be entirely excluded. The reason for such a reservation is that so far there have been limited studies on the influence of hypomagnetic conditions on tardigrade survivability, and none on other extremophilic invertebrates, such as rotifers, crustaceans or nematodes. The only papers on the influence of the magnetic field on nematodes concerns their ability to magneto-detection and magneto-navigation (Vidal-Gadea et al., 2015). Most studies on the influence of hypomagnetic conditions on terrestrial organisms, especially in the context of spaceflights, were conducted on vertebrates, that are, mice, rats and humans, or their isolated tissues (Conley, 1970; Binhi & Sarimov, 2009; Xiao et al., 2009; Fesenko et al., 2010; Binhi, 2012; Gurfinkel et al., 2016), and there were a few studies on crops/plants (Mo et al., 2011). The only organisms discovered to have higher tolerance to some environmental stressors were Bacteria or Archaea. However, even then researchers focused mostly on model organisms, such as Escherichia coli Escherich, 1885, and some pathogenic strains/species of Shigella sonnei (Levine, 1920; Weldin, 1927, Salmonella sp. or Staphylococcus sp. (Dubrov, 1978), Deinococcus radiodurans Brooks & Murray, 1981) or Leptolyngbya laminose (Gomont) (Anagnostidis & Komárek, 1988 ; Mastrapa et al., 2001; De La Vega, Rettberg & Reitz, 2007, De Vera et al., 2013). The scarcity of the above studies makes results of our experiments even more important, because they show that despite higher mortality of tardigrade species in hypomagnetic conditions, their chances to survive are not reduced to zero. It should be remembered that many species of tardigrades including Hys. exemplaris, Ech. testudo and Mil. inceptum are parthenogenetic (Nelson, Guidetti & Rebecchi, 2015), which means that even one specimen that survives a voyage in hypomagnetic conditions and finds favourable conditions can still establish a new population able to function in a normal way.
Conclusion
In conclusion, although the current knowledge on the influence of hypomagnetic conditions on mortality of cryptobiotic organisms is still limited to tardigrades, our results suggest that the presence of the magnetic field is a very important factor should be considered in further research both in the context of potential space contamination and terrestrial organisms surviving on other planets and moons.
Supplemental Information
Temperature data for experiments I–III carried out on both Ech. testudo and Mil. inceptum.
Temperature data used to calculate the average temperature inside the CIMF chamber. The table contains temperature data for 10:00 am and 10:00 pm, each day in every 24-day period (3 days of desiccation time period and 21 days of experimental time), for experiments I–III carried out on bothEch. testudo and Mil. inceptum.
Summary of raw data from experiments I–III carried out on tardigrades of the species Ech. testudo.
The data includes the number of dead individuals, the number of alive individuals and the percentage mortality of the tested replicates.
Summary of raw data from experiments I–III carried out on tardigrades of the species Mil. inceptum..
The data includes the number of dead individuals, the number of alive individuals and the percentage mortality of the tested replicates.
Comparison of experiments I–III carried out on tardigrades of the species Ech. testudo.
The data includes comparisons between all three experiments I–III and control. The control group in this case, was expressed as the minimum values for each dish from the control groups for experiment. diff, differences between groups; lwr, lower 95% confidence level; upr, upper 95% confidence level; p adj, p value.
Comparison of experiments I–III carried out on tardigrades of the species Mil. inceptum.
The data includes comparisons between all three experiments I–III and control. The control group in this case, was expressed as the minimum values for each dish from the control groups for experiment. diff, differences between groups; lwr, lower 95% confidence level; upr, upper 95% confidence level; p adj, p value.
Additional Information and Declarations
Competing Interests
The authors declare that they have no competing interests.
Author Contributions
Weronika Erdmann conceived and designed the experiments, performed the experiments, analyzed the data, prepared figures and/or tables, authored or reviewed drafts of the paper, and approved the final draft.
Bogdan Idzikowski conceived and designed the experiments, analyzed the data, authored or reviewed drafts of the paper, a crucial role in design and construction ideas of magnetic equipment (Chamber Isolated from Magnetic Field - CIMF) used in experiments, and approved the final draft.
Wojciech Kowalski conceived and designed the experiments, authored or reviewed drafts of the paper, a crucial role in technical construction of magnetic equipment with digital (computer) control, and approved the final draft.
Jakub Z. Kosicki analyzed the data, authored or reviewed drafts of the paper, and approved the final draft.
Łukasz Kaczmarek conceived and designed the experiments, authored or reviewed drafts of the paper, and approved the final draft.
Data Availability
The following information was supplied regarding data availability:
The raw data are available in Supplemental Files S1, S2, and S3. The raw data in Supplemental File S1 show temperature data from the chamber. These data were used for determining the average temperature inside the chamber. The raw data in Supplemental Files S2 and S3 show the number of dead and alive specimens in test and control groups of all replications, of each experiment. These data were used for statistical analysis to compare differences in mortality between test and control groups.
Funding
This work was supported by the Polish Ministry of Science and Higher Education via the “Diamond Grant” program (grant no: DI2012 016342), “Study of resistance of small invertebrates cryptobiotic (for example tardigrades) to the lack of the geomagnetic field and the survivability of terrestrial organisms beyond our planet.” There was no additional external funding received for this study. The funders had no role in study design, data collection and analysis, decision to publish, or preparation of the manuscript.